Time-Correlated Single Photon Counting
Highly sensitive single-photon detectors (SPD) provide valuable signals whose temporal analysis offers unprecedented possibilities in astrophysics, materials science, quantum information technology, quantum encryption, medical imaging, DNA sequencing and fiber-optic communication. For example, fluorescence lifetimes of excited matter can be deduced (FLIM). This is the perfect job for our TDCs.
Time-correlated single photon counting (TCSPC) is a widely used technique for the time-resolved measurement of fluorescence. The particularly high time resolution that is achieved today is a special feature of this technology. This method achieves the technically feasible state of the art in many aspects - e.g. individual photons are recognized. This measurement method is used as an essential part of imaging techniques such as FLIM (fluorescence lifetime microscopy). Time-Correlated Single Photon Counting is a particularly non-invasive measurement technique because the light pulses required for sample excitation have low energy. The aforementioned single-photon detection also contributes here.
In detail, TCSPC is based on the detection of the arrival times of the photons on a detector. The time of emission of the laser pulse is usually taken as the reference signal, which thus reflects the time of excitation of the sample. The sample is excited with the laser pulse at a high repetition rate. The high repetition rate is of great advantage in order to obtain a statistically significant course of the de-excitation behavior of the sample (in the shortest possible time) from the single-photon measurement. Fluctuations in the source intensity and the resulting measurement errors are also compensated for by the enormous frequency of measurements. To measure the photon emission times, TDC systems are used on the one hand and fast ADCs in the Gsps/s range on the other.
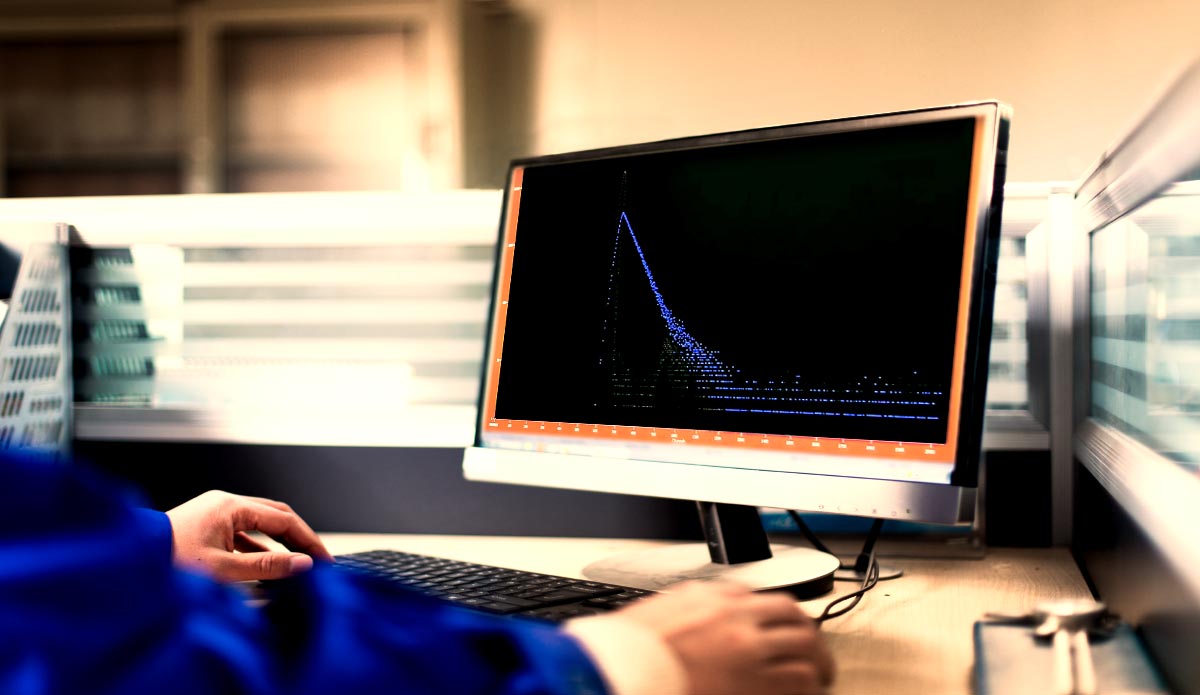

Laser sources, sometimes femtosecond lasers, serve as the source for exciting light. When choosing the laser sources, the excitation energies of the sample and its expected fluorescence lifetime play a decisive role.
With a high repetition rate (usually in the range of 1 - 100 MHz), the laser sends a start signal to a computer at the same time as the pump pulse is emitted from the source. This start pulse triggers the time measurement.
The laser pulses are typically shorter than 0.1 ns and are directed onto the sample via a neutral density filter. The filter suppresses stray light that could falsely trigger the detector and attenuates the excitation pulse accordingly. If a photon is emitted from the sample within a pulse cycle and hits the detector, this triggers the stop signal of the time measurement.
In front of the detector, the so-called cutoff filter, which limits the measurement to the relevant spectral range. In some measurement setups, the light emitted by the sample molecule passes in addition to a monochromator in order to provide information on the emitted wavelength.
The synchronization of the measurement signals and their digitization is implemented with the help of fast, high-precision data acquisition technology, which yields histograms of the recorded timing data in the end- this is the core competence of cronologic.
The following photon detectors are used for TCSPC measurements:
- PMT: (Photomultiplier Tube) - the classic approach, cheap, high gain, not good for fast detection.
- MCP: Microchannel plates are very popular because they are more sensitive than PMTs and have faster response times.
- APD / SPADs (Avalanche Photodiodes): The semiconductor equivalent to a PMT provides very short pulses (FWHM 30-400ps) and consequently enables the detection of very fast photoluminescence decays.
- Hybrid PMTs combine a classic PMT with an avalanche diode, providing a low propagation time spread and advantages in terms of counting efficiency. These detectors are practically free from post pulses. Much of the detector's gain is achieved in a single step. As a result, such detectors generate single-photon pulses with a narrow pulse height distribution. With such detectors, it is much easier to differentiate between accumulated photons. The small-amplitude fluctuation eliminates the residual influence of the CFD circuit on the time jitter.
Possible problems of TCSPC measurements
Special attention should be paid to possible measurement errors due to the effect of the so-called "pulse pile-up" during a TCSPC measurement:
If two or more photons are emitted within a very short time interval, it can happen, for example, due to dead times of the data acquisition system, that individual photons are not recorded. The resulting measurement error can systematically alter the recorded histogram since mostly the photon “arriving later” is lost. The problem of the “pile-up” of photons can be avoided by reducing the excitation intensity (and thus the percentage of photons that are emitted from the sample). Accordingly, TCSPC setups are designed in such a way that only a fraction, often only one, of 50 excitation pulses generates a fluorescence response.

TCSPC measurements: then and now
In order to register the arrival time of the fluorescence photons relative to the excitation time of the sample, electronics with the highest possible resolution are used. Initially, the TCSPC-data was recorded with the help of a TAC (Time to Amplitude Converter) and a transient recorder (Analog to Digital Converter, ADC):
The signals containing the information on the emission time are processed by a constant fraction discriminator (CFD). The reference pulse then activates the TAC. The TAC internally generates a voltage ramp on a capacitor, whereby the voltage rise is stopped as soon as the fluorescence photon from the sample is detected. The TAC voltage is then evaluated by an ADC and converted into a digital timestamp. A higher voltage equals a later emission time. In the reverse TAC mode, the roles of the excitation pulse and the detected photon are reversed. In this case, the excitation pulse stops the voltage increase in the TAC.
The recorded timestamp can be processed further in a multi-channel analyzer (MCA) for histogramming. This classic measurement method has a comparatively long dead time.
Newer TCSPC systems use the fast and efficient data acquisition concepts of modern TDCs (Time to Digital Converter/time interval analyzer), which convert the arrival time of the timing signals directly into digital timestamps. Due to the short dead times of modern TDCs, it is sometimes even possible to record several photons per excitation cycle. If necessary, additional information can be recorded for each photon detection event, e.g. the light’s wavelength, its polarization, its position within an image area, information on sample properties, the excitation wavelength, etc. This results in a manifold of further options for displaying and analyzing the measured data.
The limits of TCSPC measurements
The resolution of TCSPC measurements is limited by various factors, the sum of which is referred to as the IRF (Instrument Response Function):
- The finite width of the laser pulse (largest factor)
- finite rise/fall of electronic signals
- The time resolution of the detectors
- Jitter in electronics
The IRF time sum can be obtained typically in-situ by performing a measurement of the “prompt”.
Author: Uwe Thomaschky
At this point, we would like to give visitors to our website who are interested in the possible applications for our products a little insight. We ourselves are primarily concerned with the requirements of our customers with regard to data acquisition and are neither chemists, biologists, or medical professionals, nor do we build mass spectrometers or imaging systems. We just enjoy learning from our customers and sharing what we have learned with those who are interested in our work. The following contents are therefore not scientific treatises, but also reflect subjective impressions.