LIDAR
LIDAR Systems emit ultraviolet, visible, or near-infrared light to image objects and measuring the time-of-flight (TOF) of reflected photons. Such systems are used for object detection and tracking in many different fields, ranging from archaeology to agriculture, autonomous vehicles, and robots, etc. The high timing resolution of cronologic ADCs and TDCs is a key to reaching the highest ranging accuracy and our devices’ high data throughput allows for targeting even complex measurement scenarios.
Introduction to LIDAR Technology
LIDAR technology is based on a pulsed time-of-flight (TOF) measurement method for distance ranging and is used for the three-dimensional acquisition of coordinates with high precision and measuring point density. In this process, light in the form of a pulsed laser is used for distance measurement to obtain accurate 3-D information about an object in relation to its surroundings. In systems that are based on direct time-of-flight
(DTOF, ToF cameras, ToF image sensors) the key measurement parameter is the time of backscatter of the said light pulse, which is acquired using fast TDCs. In some LIDAR applications, particularly powerful ADCs are used instead. In terms of measured backscatter, different types of scattering are relevant for LIDAR, most commonly elastic Rayleigh scattering, non-molecular scattering (Mie scattering), Raman scattering, and fluorescence.
There are two types of LIDAR detection systems: "Incoherent" energy detection directly measures the amplitude changes of the backscattered light, so these systems require fairly high laser power. Coherent LIDAR measurements typically measure optical superpositions such as Doppler shifts or changes in the phase of the backscattered light. They allow operation with lower (eye-safe) laser power, whereby both detection and data acquisition are much more complex and require larger bandwidths.
Nowadays, the information obtained from the light pulses is usually combined with further data points obtained simultaneously, e.g. from a satellite navigation system (GNSS) and an INS (Inertial Navigation System). LIDAR devices can be used very flexibly, often they are mounted on moving objects, e.g. airplanes, vehicles, or drones, and thus serve for navigation and/or the acquisition of environmental data. However, they can also be stationary, e.g. for measuring the backscatter of gases in the monitoring of industrial plants.
The high-precision x, y, and z measurements of modern LIDAR systems provide ultra-high-resolution 3D point clouds with an accuracy in the centimeter range and are faster than RADAR or cameras, for example. They can also detect non-metallic objects at night, unlike radar or camera sensors. Depending on the technique used, LIDAR measurements offer the possibility to "see-through" liquids (such as water bodies in bathymetry) or solid objects (such as walls or trees).
The quality differences of different LIDAR systems are immense, as the technology has developed tremendously over the last decades. The LIDAR system of a smartphone cannot be comparable to a modern airborne LIDAR. Airborne LIDAR, also known as Airborne Laser Scanning (ALS), has played a crucial role in the advancement of LIDAR systems. These dynamic multi-sensor systems continuously measure the position and attitude of the sensor platform while the laser scanner provides the direction of the laser beam and the distance between the sensor and reflecting objects. For many applications, e.g. in the field of driver assistance or autonomous driving in road traffic, a relatively low point density is accepted for cost reasons. Near-infrared lasers are often used in this area. Since the infrared beams become wider with increasing distance, they offer excellent resolution at close range, but as the distance between the objects increases, the measurements become increasingly blurred. Additional sensor technology, e.g. cameras, radar, and ultrasound, is often used for these applications.
Even though autonomous driving may be a large growth market at the moment, we at cronologic focus on our core competencies - and that is the measurement of extremely high pulse repetition rates, which enable significantly higher point densities and thus enormously high resolution, especially when using single-photon sensitive receiver arrays. This is particularly advantageous in the field of airborne sensing, space technology, robotics, and automation.
Our TDCs and ADCs, therefore, score highly in those long-range high-speed application areas where high demands on resolution and resistance of measurements to interference are factored into the price-performance ratio. To understand why it is worth taking a look at the advances in LIDAR technology.
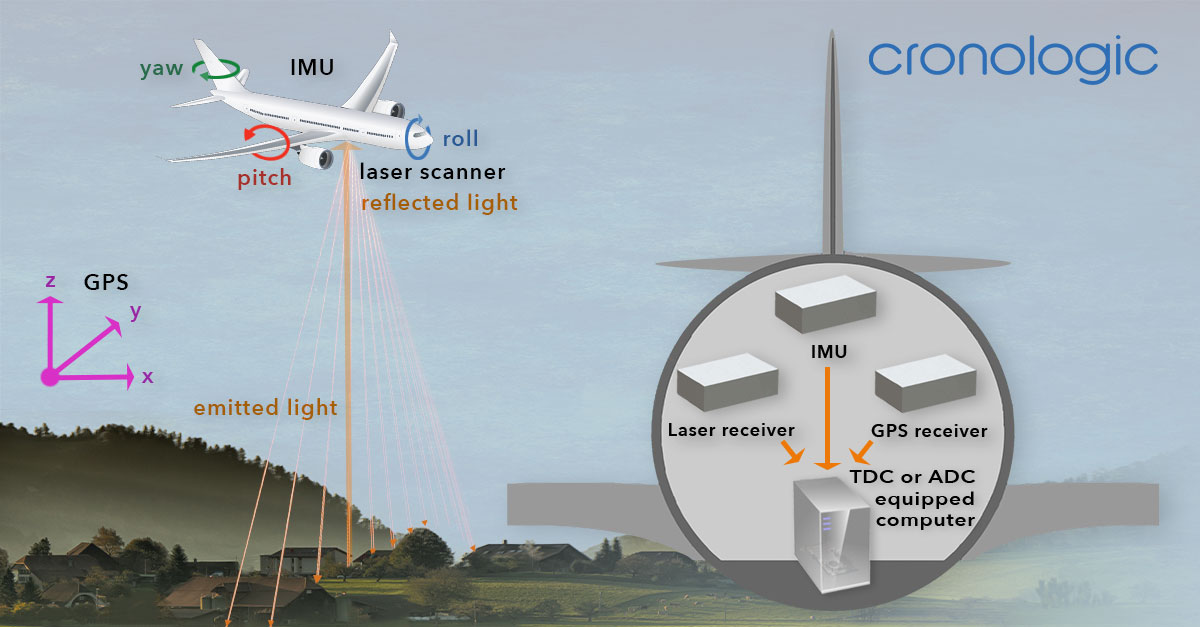

- Single-photon LIDAR (SPL) is a comparatively new and significant development. Whereas in the past several hundred photons were required to detect an echo, today single-photon sensitive sensors (e.g. SPADS or avalanche photodiodes) are used, which allow real-time scanning of larger areas at the same point density. In SPL, a collimated laser pulse simultaneously emits a large number of mutually but non-overlapping sub-beams, each of which is assigned on the detector side an individual field of view aligned to its spatial direction. As a rule, this technique uses laser radiation in the visible green range of the spectrum (wavelength λ=532 nm). In single-photon LIDAR, multiple laser pulses can be detected simultaneously and analyzed separately. This offers the possibility to increase the scan rate by already emitting new laser pulses before the return of the first pulse arrives, therefore increasing the density of the measured point cloud even further. The higher point density allows for greater flight heights and thus higher area performance, making this technology particularly attractive for acquiring and updating topographic datasets. In addition, the wavelength used offers the possibility of taking bathymetric measurements and combining them with topographic surveys. Single-photon LIDAR is also used for military reconnaissance because of the special potential of this measurement technique.
Current Trends in LIDAR
A clear trend in airborne LIDAR technology is the fusion of original data acquired by means of LIDAR with further active and passive sensor technology. This includes the simultaneous acquisition of RGB or color infrared (CIR) images or the use of multispectral cameras for documentation purposes in the context of airborne mapping, but also the combination of LIDAR techniques with Dense Image Matching (DIM). This technique aims to compute a depth value for each pixel of an image by comparing two overlapping images line by line to generate accurate and highly detailed digital surface models (DSMs). Currently, the fusion of image-derived height estimation with data obtained via LIDAR measurements is the subject of ongoing research.
DAQ in LIDAR:
The entire data processing flow from data acquisition to production output can be divided into five key stages:
- data acquisition
- data pre-processing
- filtering and classification of point clouds
- fusion and application of LIDAR data and other remote sensing data
- extraction of objects and 3D modeling based on LIDAR data.
Data acquisition is a central aspect of the development of LIDAR systems. Last but not least, the information sensed from the distance measurement must be merged with the GPS coordinates and the data packets generated by the IMU and meaningfully processed into three-dimensional point clouds and displayed accordingly. The distance (D) from the object is calculated from the equation D = 1/2 x TOF x c, where c is the speed of light through the medium. The resolution and quality of the resulting representation depend, among other things, to a large extent on the point density of the LIDAR system. Consequently, the primary goal of most LIDAR system developments has been to increase the point density of the measurements. This results in another major challenge: increasing the data throughput, especially in modern applications where the 3D data obtained from particularly short pulses via LIDAR are in some cases additionally supplemented with the two-dimensional data from a camera-based image processing system. After all, modern scanners, which often emit more than 300,000 laser pulses per second, can generate several gigabytes of LIDAR data in an hour.
Data acquisition for Linear LIDAR is still comparatively economical in terms of data volume: the discrete acquisition of individual pulses is already possible at low sample rates. With full-waveform LIDAR, a complex waveform is acquired and provides a more detailed image, which means that the data volumes are significantly higher. Depending on the application, the captured waveforms are either processed directly in the sensor's firmware or initially stored for detailed analysis in post-processing. In the Geiger-mode LIDAR method, returns from a larger field of view are acquired from different angles. Accordingly, the sample rate is quite high (e.g. in the order of 200 MS/s) and the point density is also increased accordingly. The resulting data sets are correspondingly large. In order to reduce measurement noise and estimate signal intensity, redundant echoes from the largely overlapping laser scans must also be analyzed in post-processing, so that imaging cannot usually be performed in real-time. And with single-photon LIDAR, the particularly high density of the collected data naturally leads to very large data sets.
The above-mentioned point cloud is a complex mapping of 3D space that needs to be categorized and understood. Therefore, in many applications, the measured points are often read as polygons, because the resulting shapes are easier for computers to process. Since not all points of a measured object are detected by LIDAR, the detection rate (DR), also called true-positive rate (TPR), is a crucial quality criterion. This describes the proportion of images in which a selected point on a real target is detected. This criterion is supplemented by the false positive rate (FPR), which describes the proportion of images in which an echo is detected in the point cloud although no real physical target is present.
Regarding the accuracy of LIDAR systems, the quality of the TDCs (or ADCs) used has a huge impact. Distance measurement with a high TPR and the lowest possible FPR can be achieved above all by keeping the probability of trigger errors as low as possible. Extensive simulations and tests have shown that a good signal-to-noise ratio in the data acquisition hardware is crucial for an acceptable error probability. If a high distance precision is to be achieved by means of direct TOF measurement (as shown in the first figure), then the time jitter of this measurement must be kept as small as possible, because this directly affects the distance precision of the measured point cloud.
LIDAR Applications
Originally, LIDAR was used for atmospheric research and meteorology. LIDAR measurements are applied in many areas and are currently finding their way more and more into our everyday lives, especially in the course of e-mobility, gaming, and autonomous driving. Probably one of the best examples of this is the integration of LIDAR sensors in various smartphones and household robots.
At this point, we would like to give a brief overview of the rather more sophisticated LIDAR applications. Since we offer cronologic products exclusively for civilian purposes, we leave out the military applications:
- Topography & Cartography: In Topographic LIDAR, landmasses are usually mapped with a near-infrared laser. Today, airborne LIDAR enables precise, area-wide 3D data acquisition and, in contrast to camera systems, is not influenced by the shadow angle of the sun. Thus, digital terrain models (DTM) or elevation models (DEM) of topography, vegetation, and infrastructure can be created.
- Land management, urban and regional planning: Airborne LIDAR enables the real-time study of the evolution of geographic space and faster and more efficient high-resolution mapping for the creation of geographic information systems (GIS). These form the basis for numerous early warning systems, disaster preparedness, and the implementation of various emergency measures.
- Geology: LIDAR data allow geologists to study the topology of the Earth and its formation in the context of geomorphology.
- Bathymetry: Bathymetric LIDAR measures the seafloor and riverbed with green light penetrating the water. Bathymetric LIDAR facilitates the collection of geospatial data of the coastline and shallow waters, making the production of hydrographic data particularly efficient. With the sea-level rise and the increase in extreme natural disasters, this application is currently becoming more and more important. For example, LIDAR helps to model and predict the extent of an approaching tsunami.
- Radio Signal Mapping: In the context of wireless communication system planning, LIDAR has emerged as an alternative to digital orthophotography, facilitating the planning of radio transmitter positioning, including the evaluation of signal strength and radius of radio signals.
- Astronomy and spaceflight: Whether for measuring the distance between the Earth's surface and the lunar surface, for creating topographic maps of Mars, or for autonomous, safe precision landings of manned lunar landing vehicles - LIDAR is a key technology for space exploration.
- Meteorology: Ever since the first LIDAR systems, which were still based on elastic backscatter, LIDAR has helped meteorologists to study cloud patterns. DIAL (Differential Absorption LIDAR) is used to identify specific forms of gases in the atmosphere, while Raman LIDAR measures concentration and Doppler LIDAR measures wind speed.
- Environmental Monitoring & Atmospheric Physics: LIDAR can be used to study the molecular density of atmospheric gases, aerosols, and clouds. The accuracy of such measurements enables the detection of airborne and waterborne particles and substances such as volcanic sulfur dioxide, atomic mercury, ozone, carbon dioxide, and methane. This is useful for monitoring air quality over urban or industrial areas, weather forecasting, and climate modeling.
- Renewable energies: LIDAR systems determine wind speed higher, farther, and faster than conventional measuring masts, enabling effective site selection for wind farms. In addition, LIDAR is used on the nacelles of wind turbines to calculate the direction and strength of the wind and, if necessary, to change the direction of the rotor blades. This maximizes the efficiency of these turbines and protects them from damage.
- Climate research: Scientists use airborne LIDAR systems to study long-term changes in the atmosphere. This applies, for example, to cloud statistics for investigating the relationship between cloud formation and climate change, documenting changes in forest areas, and accurately recording the melting process of glaciers. Of particular note in this context is the Australian Antarctic Science project, in which extensive aerosol data are collected over the Southern Ocean.
- Power supply: Airborne LIDAR enables the inspection of power lines by using the measured point cloud to check the course of the lines themselves and the distance between poles. The detailed analysis of the structure of the power line corridor helps to identify potential risks and, if necessary, to carry out rapid damage analyses.
- Agriculture and forestry: LIDAR is used to analyze vegetation growth and map yield rates on agricultural land. In agriculture, for example, this can be used to determine where fertilizer needs to be applied to maximize yield and reduce fertilization to the bare minimum. Autonomous agricultural equipment today rarely does without LIDAR control, and more recently LIDAR has even been used to monitor insects in agricultural fields. Because some LIDAR systems can penetrate layers of vegetation, they are used in precision forestry to create 3D models to monitor the impact of human activities.
- Fisheries biology: the National Oceanic and Atmospheric Administration have developed a special airborne LIDAR instrument that uses images similar to those from acoustic echo sounders to display the spatial distribution of fish and for vertical profiles of fish biomass.
- Mining and ore extraction: Airborne LIDAR using drones is often used for fast and cost-effective surveying of mines, although terrestrial mobile laser scanners are of course also used for this purpose. In this way, material quantities can be calculated in open-pit mining without disrupting work on the ground.
- Oil and gas exploration: Differential Absorption LiDAR (DIAL), which is currently under development, is expected to simplify the search for oil and gas deposits.
- Archaeology: LIDAR helps archaeologists identify microtopographic patterns that are not visible from the ground and with simple aerial imagery as they are obscured by trees and shrubs, for example.
- Robotics: LIDAR is used to provide robots with orientation and navigation functions. In self-driving vehicles, LIDAR is used within an autonomous system to detect the distance between the vehicle and other objects in the environment.
Credits:
Many thanks to Dr. Gottfried Mandlburger, Senior researcher at Vienna University of Technology, Austria for his support of this article.
Image Sources:
point cloud of Chicago, Illinois: Photographer: Jason Stoker, Image rights: public domain, picture has been cropped and taken from: https://www.usgs.gov/media/images/geiger-mode-lidar-over-chicago-il
Flying over the Brazilian Amazon by NASA, Creative Commons, public domain, find more on: https://svs.gsfc.nasa.gov/10757
Author: Uwe Thomaschky
Remark:
At this point, we would like to give visitors to our website who are interested in the possible applications of our products a little insight. We ourselves are primarily concerned with the data acquisition requirements of our customers and do not build any LIDAR-Systems. However, the following content is not to be understood as a scientific treatise but also reflects subjective impressions. Part of the content of this article was inspired by Dr. Gottfried Mandlburger, Senior researcher at Vienna University of Technology and his article for GIM international on airborne LIDAR.
In this context, we would like to point out that cronologic products are not intended for military use.